Research Overview
Our research involves new synthetic method development and their application to the synthesis of structurally novel and biologically active natural products. Metal-catalyzed reactions to merge alkenes and alkynes to construct multiply unsaturated molecular structures are one of the major research interests. Aryne formation from tri- and tetraynes followed by exploiting their reactivity to develop novel transformation is another active area of our research. The reaction between lithiated trimethylsilyldiazomethane
and unsaturated carbonyl compound followed by proteolytic cleavage of N–N bond constitutes a formal 1,2-aminocyanation, which is under investigation for the construction of amino quaternary carbon-containing molecules including amathspiramides and massadine.
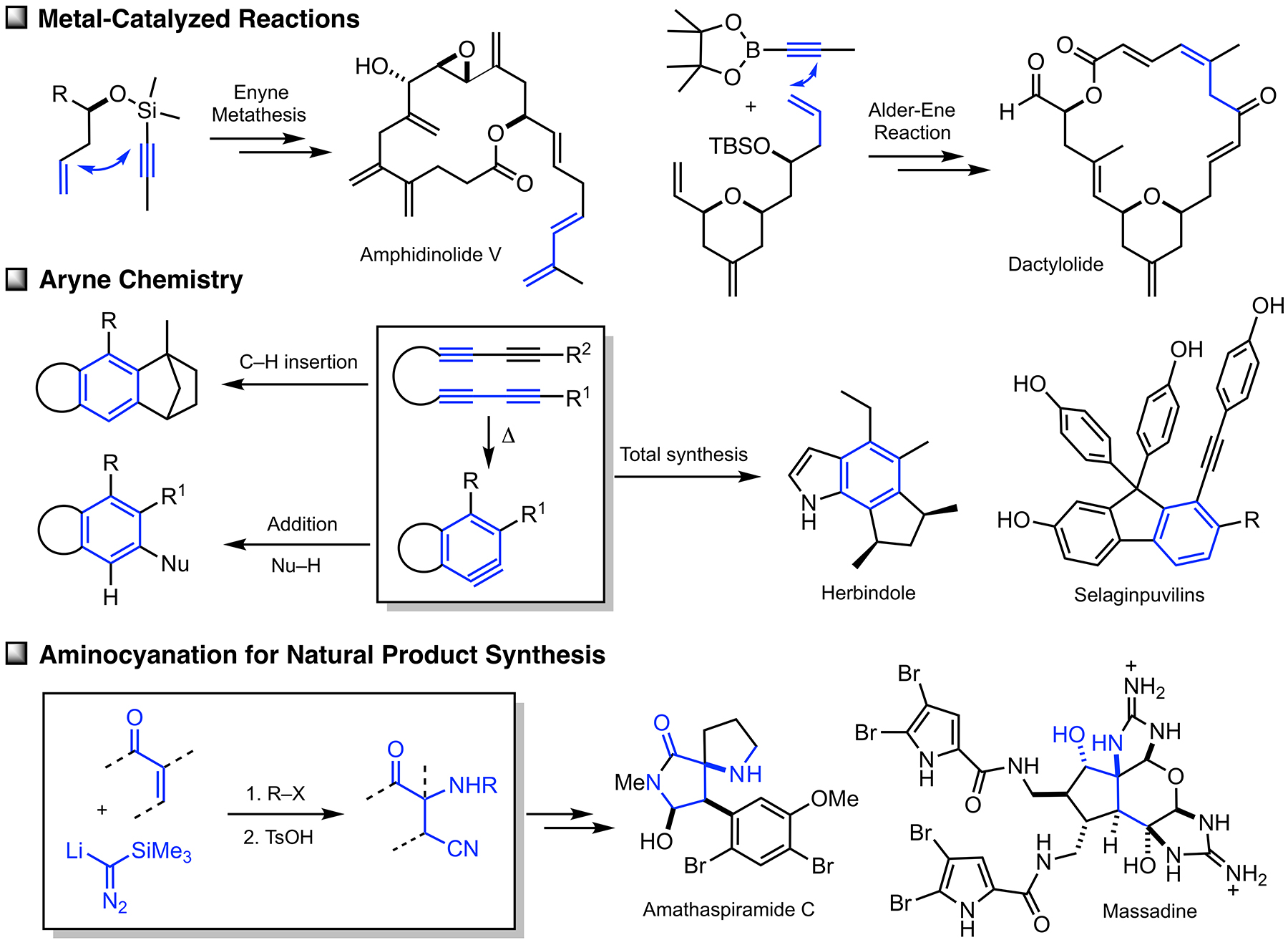
Current Research Interests
Ruthenium-Catalyzed Reactions
Olefin metathesis has become one of the most powerful synthetic arsenals for carbon-carbon formation and the impact of which has been recognized by the 2005 Novel prize in chemistry “For the development of the metathesis method in organic synthesis”. Among metal-carbene complexes that can catalyze the metathesis reactions, ruthenium-alkylidene carbenes known as “Grubbs catalyst” have shown wide range of application in organic synthesis due to their relatively
high stability to air and moisture as well as polar functional groups. Most pronounced applications of metathesis chemistry range from synthesis of natural products, pharmaceuticals, and polymers of novel properties. Additionally, the greener nature of metathesis compared to other synthetic methods has additional benefits especially considering the environmental issues. While many different types of metathesis involving alkenes such as ring-closing metathesis (RCM), cross metathesis (CM), ring-opening metathesis
(ROM), ring-rearrangement metathesis (RRM), ring-opening metathesis polymerization (ROMP), and non-metathetic transformations catalyzed by ruthenium carbenes (G-I, G-II, GH-II) have been studied extensively, the corresponding metathesis involving both alkenes and alkynes known as enyne metathesis have been underdeveloped. However, significant advances in RCM and its extension to metallotropic [1,3] shift bodes well for the further development of enyne metathesis.
[Top]
Arguably, the first enyne RCM reaction was reported by Katz and coworkers in 1985 using tungsten Fisher carbene complexes. Since then a variety of low-valent transition metals have been used to catalyze this reaction. However, it was not until the development of ruthenium carbene in the 1990’s that the enyne metathesis is considered to be a viable metathesis tool, yet it still has a narrow substrate scope due to its mechanistic complexity compared to alkene metathesis.
In light of this, we have been exploring the tandem bond-forming capacity of enyne metathesis. These endeavors have led to significant advances in our understanding of the reactivity and selectivity of various substrate platforms in RCM and CM reactions. Furthermore, the highly effective metallotropic [1,3] shift of propargylic ruthenium carbene allows for a series of sequential enyne metathesis, which can form molecules of extended conjugation with both double and triples bonds. Tandem metathesis of multiynes
with a sequence of enyne metathesis and metallotropic [1,3] shift has led to the discovery of many unprecedented transformations and hidden catalytic activities of Ru-alkylidene carbenes. These novel transformations include the formation of highly conjugated oligo-enynes (A), 1,4-hydrovinylative cyclization of triynes and tetraynes (B), benzyne formation via the hexedehydro Diels-Alder reaction of multiynes followed by transfer of hydrohalogen from common halogenated organic solvents (C),
and metallotropic shift-driven
formation of ruthenium-alkyne chelate (D). The prowess of the tandem bond-forming nature of enyne metathesis in tandem with metallotropic 1,3-shift has been applied to the synthesis of 1,3-diyne- and 1,3-enyne-containng natural products (E, F). Also, relying on enyne RCM and CM as the key step for the construction of 1,3-diynes, an efficient total synthesis of amphidinolide V was achieved (G). Our current research focuses on the development of new ruthenium alkylidene complexes to
further expand and discover
novel reactivities of these complexes and their application to the synthesis of natural products and conjugated oligomers.
[Top]
Representative Publications
- Mansuk Kim and Daesung Lee “Metathesis and Metallotropy: A Versatile Combination for the Synthesis of Oligoenynes” J. Am. Chem. Soc. 2005, 127, 18024–18025.
- Mansuk Kim, Reagan L. Miller and Daesung Lee “Cross and Ring-Closing Metathesis of 1,3-Diynes: A Facile Metallotropic [1,3]-Shift” J. Am. Chem. Soc. 2005, 127, 12818–12819.
- Sangho Park, Mansuk Kim and Daesung Lee “Regio- and Stereoselectivity Control in Cross Enyne Metathesis of Silylated Internal Alkynes” J. Am. Chem. Soc. 2005, 127, 9410–9415.
- Mansuk Kim and Daesung Lee “Advances in the Metallotropic [1,3]-Shift of Alkynyl Carbenoides” Org. Biomol. Chem. 2007, 5, 3418–3427.
- Sang Young Yun, Kung-Pern Wang, Mansuk Kim, and Daesung Lee “Nonmetathetic Activity of Ruthenium Alkylidenes Complexes: 1,4-Hydrovinylative Cyclization of Multi-Ynes with Ethylene” J. Am. Chem. Soc. 2012, 134, 10783–10786.
- Rajdip Karmakar, Kung-Pern Wang, Sang Young Yun, Phani Mamidipalli and Daesung Lee “Hydrohalogenative aromatization of multiynes promoted by ruthenium alkylidene complexes” Org. Biomol. Chem. 2016, 14, 4782–4788.
- Eun Jin Cho and Daesung Lee “Total Synthesis of (3R,9R,10R)-Panaxytriol via Tandem Metathesis and Metallotropic [1,3]-Shift as a Key Step” Org. Lett. 2008, 10, 257–259.
- Jingwei Li, Sangho Park, Reagan L. Miller and Daesung Lee “Tandem Enyne Metathesis–Metallotropic [1,3]-Shift for a Concise Total Syntheses of (+)-Asperpentyn, (-)-Harveynone, and (-)-Tricholomenyn A” Org. Lett. 2009, 11, 571–574.
- Kung-Pern Wang, Sang Young Yun, Daesung Lee and Donald J. Wink “Structure and Reactivity of Alkyne-Chelated Ruthenium Alkylidene Complexes” J. Am. Chem. Soc. 2009, 131, 15114–15115.
- Sang Young Yun, Mansuk Kim, Daesung Lee and Donald J. Wink “Structure and Reactivity of Alkynyl Ruthenium Alkylidenes” J. Am. Chem. Soc. 2009, 131, 24–25.
- Ivan Volchkov and Daesung Lee “Asymmetric Total Synthesis of (–)-Amphidinolide V through Effective Combinations of Transition Metal and Organocatalysis” J. Am. Chem. Soc. 2013, 135, 5324–5327.
Aryne Chemistry
On the basis of the facile formation of halogen-containing arenes directly acyclic tetraynes, we have started to explore the reactivity of arynes generated via the hexadehydro Diels-Alder reaction toward various nucleophiles with both intra- and intermolecular reaction manifolds. Our investigation on the aryne reactivity includes reactions with metal-catalyzed addition of poor nucleophiles and those with relatively good nucleophiles without a catalyst. Representative examples of these
transformations we have explored are briefly summarized below.
[Top]
Metal-catalyzed reactions of arynes: The transformations of arynes catalyzed by a transition metal catalyst include; (A) C–H insertion, (B) inter and intramolecular hydroarylation, (C) intramolecular hydride transfer from a tethered trialkylsilyl group, (D) hydrohalogenation reactions using halogenated hydrocarbons as the source of HX, (E) hydrofluorination using pyridine-HF complex as the source of hydrogen fluoride, (F) mono-addition
of nitrile followed by trapping of water to form
amides and/or double addition of nitriles to form quinazolines, (G) double addition of isonitriles to generate benzocyclobutene-1,2-diimines, and (H) mixed addition of nitrile and isonitrile to generate 3H-indol-3-imines and 3-iminoisoindolin-1-ols.
[Top]
Thermal reactions of arynes: Due to the bond distortion of the alkyne moiety in aryne species, they readily participate in a variety of reactions, including Diels-Alder reaction, Alder-ene reactions, 1,3-dipolar cycloadditions, and nucleophile addition reactions. The representative reactions of arynes generated via the hexadehydro Diels-Alder reactions without using any catalyst involve; (I) Type-I and Type-II Alder-ene reactions, (J) intermolecular Alder-ene reaction
with functionalized
alkenes, (K) addition of α,β-unsaturated aldehydes, (L) steric pressure-driven ene-reaction to form benzocyclobetenes, (M) aryne-mediated deraromatization, (N) regioselective addition of weak nucleophiles such as alcohols, carboxylic acids, and amines, and (O) addition of nucleophiles containing a silver counter cation including AgBF4, AgCF3, AgSCF3, and AgO2CCF3 to afford the corresponding addition products and phenols. We are expanding these aryne-based addition reactions
with other nucleophiles.
[Top]
Application of aryne hydrohalogenation to the synthesis of herbindole B: The prowess of aryne-based construction of functionalized arenes was demonstrated by the synthesis of an antifeedant and cytotoxic natural product herbindole B. The key strategy for the construction of the core structure of herbindole B is the mode-selective HDDAR followed by hydrobromination of an aryne intermediate formed from bis-1,3-diyne A1. Bromoarene product A2 was converted to A3
via a three-step sequence
involving removal of the tertiary alcohol moiety, hydrogenation, and desilylation of the benzylic trimethylsilyl group. The Sonogashira coupling between A3 and 3-butyn-2-ol followed by acetylation furnished A4. Gold-catalyzed rearrangement of the acetate moiety followed by the Nazarov cyclization afforded A5. The required methyl group was installed via the addition of MeMgI followed by acid-catalyzed dehydration generated indene derivative, which was hydrogenated using the Crabtree catalyst
to provide known compound
A6. Sato achieved a total synthesis of herbindole B from A6 in two steps, thus a formal synthesis of herbindole B was achieved. Relying on the effectiveness of forming functionalized indolines by arynes, we are pursuing the total synthesis of other indole-based natural products.
[Top]
Aryne-based approach to the synthesis of selaginpulvilins: In our effort to illustrate the effectiveness and utility of aryne-based arene synthesis, selaginpulvilins were selected as a synthetic target. From a biological activity standpoint, selaginpulvilins are highly valuable targets because they show remarkable inhibitory activities against PDE4, thus these natural products can be used as a platform for developing selective PDE4 inhibitors. The synthesis of selaginpulvillins
is commenced
with a Sonogashira coupling of commercially available aldehyde B1 with ethynyltrimethylsilane to generate B2. Merging B2 and B3 followed by desilylation provided triyne B4. A Cadiot-Chodkiewicz reaction with a proper terminal alkyne afforded tetrayne B5. Oxidation of the secondary alcohol in B5 with MnO2 induced a spontaneous formation of an aryne intermediate, which was hydrogenated by cyclooctane, delivering the core of selaginpulvillins B6. Because direct Friedel-Crafts
arylation of fluorenon with phenol failed
with B6, 4-methoxyphenylmagnesiumbromide was added to the bis-benzylic ketone to generate the corresponding tris-benzylic alcohol B7. Lewis acid-mediated arylation of the tertiary alcohol was found to be problematic with B7-a containing an acetoxy group at the benzylic position, which yielded bis-phenol adduct B8 in low yield. On the other hand, desired Friedel-Crafts arylation occurred with B7-b containing a trimethylsilylmethyl substituent, which delivered B9 in good yield. Removal
of the trimethylsilyl group
afforded O-trimethyl-protected form of selaginpulvilin C, and further treatment of this compound with MeMgI (neat) at elevated temperature (160 °C) finally afforded selaginpulvilin C. The first total synthesis of this natural product (9-step, 22% overall yield) clearly illustrates the prowess of HDDAR as the tool to construct highly elaborated fluorene framework from of a relatively simple acyclic multiynes. We are currently pursuing the synthesis of other selaginpulvilins from B9.
[Top]
Representative Publications
- Sang Young Yun, Kung-Pern Wang, Nam-Kyu Lee, Phani Mamidipalli and Daesung Lee “Alkane C–H Activation by Aryne Intermediates with a Silver Catalyst” J. Am. Chem. Soc. 2013, 135, 4668–4671.
- Nam-Kyu Lee, Sang Young Yun, Phani Mamidipalli, Ryan M. Salzman and Daesung Lee, Tao Zhou, and Yuanzhi Xia “Hydroarylation of Arynes Catalyzed by Silver for Biaryl Synthesis” J. Am. Chem. Soc. 2014, 136, 4363–4368.
- Phani Mamidipalli, Sang Young Yun, Kung-Pern Wang, Tao Zhou, Yuanzhi Xia, and Daesung Lee “Formal Hydrogenation of Aryne with Silyl Cβ–H Bonds as an Active Hydride Source” Chem. Sci. 2014, 5, 2362–2367.
- Kung-Pern Wang, Sang Young Yun, Phani Mamidipalli and Daesung Lee “Silver-Mediated Fluorination, Trifluoromethylation, and Trifluoromethylthiolation of Arynes” Chem. Sci. 2013, 4, 3205–3211.
- Sourav Ghorai and Daesung Lee “Aryne Formation via the Hexadehydro Diels-Alder Reaction and Their Ritter-type Transformations Catalyzed by a Cationic Silver Complex” Tetrahedron 2017, 73, 4042–4069.
- Rajdip Karmakar, Phani Mamidipalli, Sang Young Yun, and Daesung Lee “Alder-Ene Reactions of Arynes” Org. Lett. 2013, 15, 1938–1941.
- Saswata Gupta, Piepie Xie, Yuanzhi Xia, and Daesung Lee “Reactivity and Selectivity in the Intermolecular Alder-Ene Reactions of Arynes with Functionalized Alkenes” Org. Lett. 2017, 19, 5162–5165.
- Rajdip Karmakar, Sourav Ghorai, Yuanzhi Xia, and Daesung Lee “Synthesis of Phenolic Compounds by Trapping Arynes with a Hydroxy Surrogate” Molecules 2015, 20, 15862–15880.
- Rajdip Karmakar, Anh Le, Peipei Xie, Yuanzhi Xia, and Daesung Lee “Aryne-mediated Dearomatization of Arylsulfonyl Group” Org. Lett. 2018, 20, 4168–4172.
- Rajdip Karmakar Daesung Lee “Total Synthesis of Selaginpulvilin C and D Relying on in situ Formation of Arynes and Their Hydrogenation” Org. Lett. 2016, 18, 6105–6107.
- Saswata Gupta, Yongjia Lin, Yuanzhi Xia, and Daesung Lee “Aryne–Based Intramolecular Alder-Ene Reaction to Form Benzocyclobutenes Driven by High Strain Energy and Steric Pressure to Overcome a Thermodynamic and Kinetic Barrier” Chem. Sci. 2019, 10, 2212–2217.
New Reaction Development with Trimethylsilyldiazomethane
Trimethylsilyldiazomethane (TMSCHN2) has been engaged in various synthetic transformations and we have been exploring the novel reactivity of TMSCHN2 especially in carbon-carbon bond forming reactions, which are graphically outlined. As opposed to a common Lewis acid-catalyzed one-carbon homologation withTMSCHN2, the corresponding lithiated reagent TMSC(Li)N2
is a much stronger nucleophile, thus it reacts readily with a broader range carbonyl compounds to generate one-carbon
homologation product with high selectivity (Path A). On the other hand, the same intermediate can undergo an intramolecular cyclization with tethered alkene to generate Δ1-pyrazolines (Path B). By controlling the reaction temperature, the adducts between carbonyl compounds and TMSC(Li)N2 undergo elimination of LiOSiMe3 followed by N2 to generate alkylidene carbenes. This highly reactive
unsaturated carbene species can participate in an
insertion reaction with
a C–H bond to generate cyclopentene
derivatives (Path
C). The insertion of alkylidene carbene onto a bridgehead C–H was also possible, which was employed as a key strategy in the novel construction of platensimycin core. α-Silyl ketones react with TMSC(Li)N2 to generate an alkylidene carbene intermediate, which readily undergo C–Si bond insertion to generate silyl cyclopropenes, treatment of which with PtCl2 efficiently rearrange the cyclopropene moiety to generate silyl
allenes (Path D). Alkylidene carbenes
generated from γ,δ-unsaturated ketones
undergo addition
onto the π-bond to generate highly strained bicyclo[3.1.0]hex-1-ene systems, which readily undergo dimerization (Path E). It was discovered that alkylidene carbenes generated from various acyclic and cyclic ketones readily react withTMSCHN2, providing silyl allenes, which are prone to react with molecular oxygento generate the corresponding propargylic peroxides (Path F). α,β-Unsaturated cyclic ketones react with TMSC(Li)N2
in a 1,4-addition mode followed
by cyclization to generate pyrazolines,
which lead to
non-reductive N–N bond cleavage to generate α,β-aminocyanation products upon treating with TsOH (Path G). These α,β-unsaturated cyclic ketones also react sequentially with 2 equivalents of TMSC(Li)N2. With appropriate structural elements, alkylidene carbenes generated from lithium pyrazolinate intermediate can undergo fragmentation to form pyrazoles containing a tethered alkyne moiety, or alternatively undergo N–L bond insertion followed by cycloreversion
of the azete intermediate
to provide 1,2-diazepines
(Path H).
[Top]
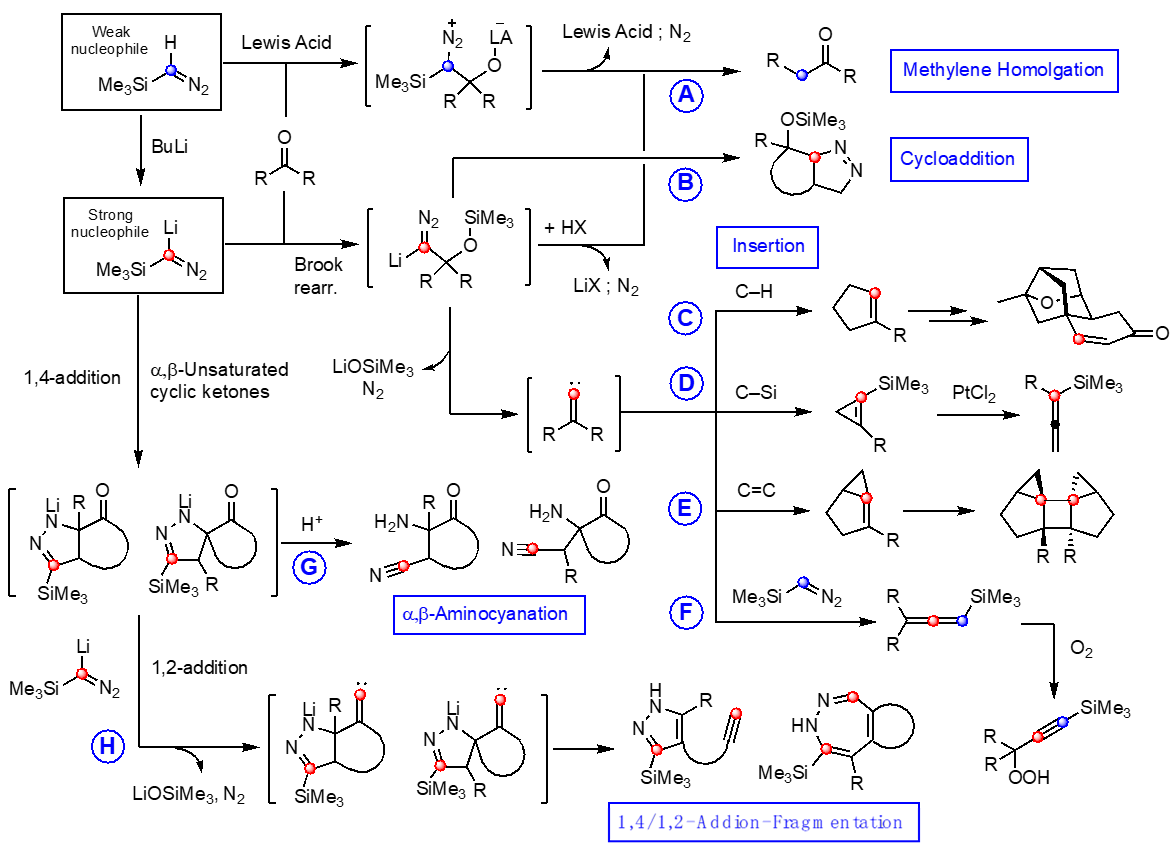
Alkylidene carbene insertion for the synthesis of platensimycin: The fate of alkylidene carbenes depend on the interplay of multiple parameters. One of the ways to steer the alkylidene carbene insertion toward a specific C–H bond is to install an activating group such as an ether functionality to the carbon bearing the C–H bond undergoing the insertion. In general, the activating role of the oxygen can be rationalized by the participation of its lone-pair electrons. However,
the
extent
of relative contribution by oxygen substituents in different environments and the exact mechanism by which these oxygen substituents activate a particular C–H bond toward the insertion are not known. In this regard, we hypothesized that the effect of the oxygen substituent should be manifested by the n(O) to σ*(C–H) electron delocalization. If this is indeed the case, insertion reactions with conformationally constrained systems would unambiguously reveal the stereoelectronic effect of the oxygen,
thereby the
regio- and stereoselectivity of these reactions will become predictable. This hypothesis was tested by employing probes C1 and C3. As shown, the lone-pair electrons in C1 would activate the axial C–Ha bond, leading to insertion product C2. On the other hand, in C3, due to the poor alignment of the orbitals, the expected n(O) to σ*(C–H) delocalization will be minimal if not zero, thus the oxygen functionality is an electron-withdrawing group because of its strong inductive
effect.
In this situation, the Ha will
become deactivated to the insertion, which would direct the insertion to Hb to generate C4. Indeed, C1 gave exclusively C2, whereas C3 afforded product C4. This strong stereoelectronic effect-based selectivity control of C–H insertion guided us to design a synthesis strategy for platensimycin.
[Top]
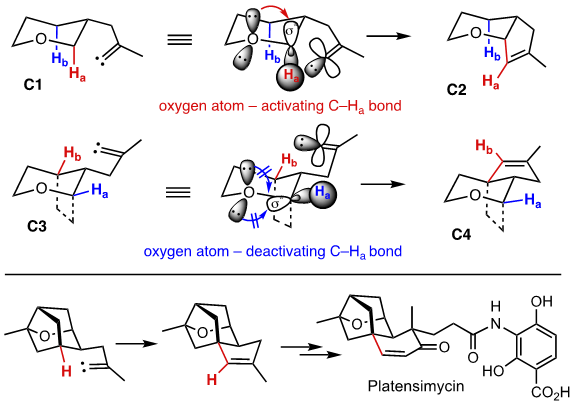
Based on this plan, we prepared bridged oxabicycle D1 starting from reducing (S)-carvone (LAH, –78 °C) and bromoetherification (NBS, –78 °C). The diastereoselectivity for these two steps are 15:1 and 12:1 respectively. The stereochemistry at the newly formed quaternary center was confirmed by an X-ray crystallographic analysis of D2, which was obtained via allylic oxidation of the methyl group (SeO2 followed by
PCC). Cyclization of bromoaldehyde D2 was accrued out by Bu3SnH/AIBN at 65 °C (81%, 4.5:1). One-carbon homologation of D3 via the Wittig reaction and hydrolysis with NBS/zinc powder provided the homologated aldehyde D4. Addition of methyl Grignard reagent followed by oxidation afforded ketone D5. Treatment of ketone D5 with TMSC(Li)N2 generated from BuLi andTMSCHN2
afforded
C–H insertion product D6. (KOH in Dihydroxylation of D6, oxidative cleavage of the resulting diol (NaIO4),
and intramolecular aldol/dehydration
MeOH) provided enone D7 in excellent yield. Following the protocol reported by Nicolaou, the tricyclic core D7 was elaborated to platensimycin. This C–H insertion strategy is currently exploited for the synthesis of other terpene natural products including lungshengenin D, which contain the identical caged structure D7.
[Top]
Aminocyanation of α,β-unsaturated esters for the synthesis of amathaspiramides and massadine: α-Amino ketones constitute an important class of biologically active natural products and pharmaceuticals. Conceptually, one of the most straightforward synthetic approaches to the α-amino ketone is α-amination, wherein an enol or enolate of ketones reacts with an electrophilic nitrogen-containing species. Various α-amination methods
have been developed, yet most of these methods share
a typical
shortcoming, which is the tedious manipulation of removing the extra functionality on the nitrogen after C–N bond formation. In this regard, we demonstrated that the addition of TMSC(Li)N2 with α,β-unsaturated ketones to form Δ2-pyrazolines followed by facile proteolytic N–N bond cleavage as an effective method to introduce a free amino group at the α-position of cyclic ketones. This formal aminocyanation reaction
of ketones could be extended to α,β-unsaturated
esters, which enables the preparation of various
structurally novel α-amono esters that contain a cyano group at the β-position. This simple protocol has a significant merit compared to the traditional amination, especially in terms of cleaving a relatively strong N–N bond under non-reductive conditions
[Top]
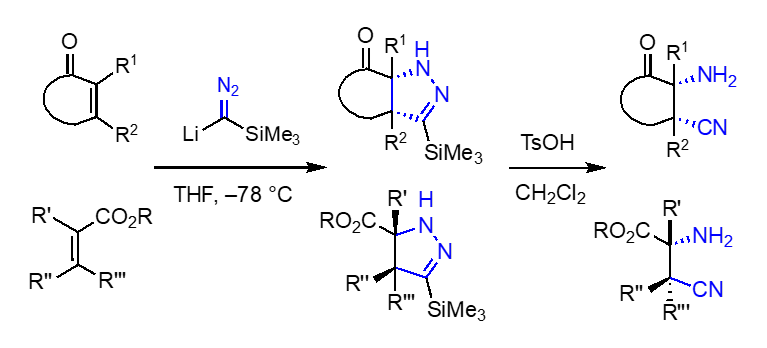
Relying on this aminocyanation, novel strategies for the synthesis of the amathaspiramides and massadine were developed. These synthetic approaches embrace the de novo construction of the quaternary carbon center containing a C–N bond by 1,4-addition of TMSC(Li)N2 with α,β-unsaturated esters. The synthesis of amathaspiramide C and the pivotal intermediate that lead to all members of the amathaspiramide family as well as the core of
massadine
have been achieved relying on the aminocyanation.
Currently, our effort is directed to the completion of massadine total synthesis.
[Top]
Representative Publications
- Sang Young Yun, Jun-Cheng Zheng and Daesung Lee “Stereoelectronic Effect for the Selectivity in C–H Insertion of Alkylidene Carbenes and Its Application to the Synthesis of Platensimycin”J. Am. Chem. Soc. 2009, 131, 8413–8415.
- Jingwei Li, Chunrui Sun, and Daesung Lee “Cyclopropenation of Alkylidene Carbenes Derived from α-Silyl Ketones”J. Am. Chem. Soc. 2010, 132, 6640–6641.
- Jingwei Li, Chunrui Sun, Silviya Demerzhan, and Daesung Lee “Metal-Catalyzed Rearrangement of Cyclopropenes to Allenes”J. Am. Chem. Soc. 2011, 133, 12964–12967.
- Jun-Cheng Zheng, Sang Young Yun, Chunrui Sun, Nam-kyu Lee and Daesung Lee “Selectivity Control in Alkylidene Carbene-Mediated C–H Insertion and Allene Formation” J. Org. Chem. 2011, 76, 1086–1099.
- Huaqing Liu, Matthew J. O’Connor, Chunrui Sun, Donald J. Wink, and Daesung Lee “Sequential Reactions of Trimethylsilyldiazomethane with Carbonyls & Tethered Alkenes Catalyzed by Lewis Bases” Org. Lett. 2013, 15, 2974–2977.
- Chunrui Sun, Matthew J. O’Connor, Daesung Lee, Donald J. Wink, and Robert D. Milligan “Formal Aminocyanation of α,β-Unsaturated Cyclic Enones as an Efficinet Entry to the Synthesis of a-Amino Ketones” Angew. Chem., Int. Ed. 2014, 53, 3197–3200.
- Jun-Cheng Zheng, Huaqing Liu, Nam-Kyu Lee, and Daesung Lee “Dimerization Behaviour of Substituted Bicyclo[3.1.0]hex-1-ene Derivatives” Eur. J. Org. Chem. 2014, 506–510.
- Matthew J. O’Connor, Chunrui Sun, and Daesung Lee “Synthesis of Amathaspiramides by Aminocyanation of Enoates” Angew. Chem., Int. Ed. 2015, 54, 9963–9966.
- Chunrui Sun, Hyunjin Lee, and Daesung Lee “Synthesis of the Carbocyclic Core of Massadine” Org. Lett. 2015, 17, 5348–5351.
- Matthew J. O’Connor, Chunrui Sun, Xinyu Guan, Venkata R. Sabbasani, and Daesung Lee “Sequential 1,4-/1,2-Addition of Lithiumtrimethylsilydiazomethane onto Cyclic Enones to Induce C–C Fragmentation and N–Li Insertion” Angew. Chem., Int. Ed. 2016, 55, 2222–2225.
- Yuanzhi Xia, Nam-Kyu Lee, Venkata R. Sabbasani, Saswata Gupta, and Daesung Lee “Activation of Vinylic over Allylic C–H Bond in Silylallenes with Molecular Oxygen: A New Perspective on C–H Bond Strength” Org. Chem. Front. 2018, 5, 2542–2546.
|